Journal of Physical Studies 26(4), Article 4702 [10 pages] (2022)
DOI: https://doi.org/10.30970/jps.26.4702
PROPERTIES OF ELECTRON STATES IN A CLOSED MULTI-CASCADE NANOSTRUCTURE BEING AN ELEMENT OF A QUANTUM CASCADE DETECTOR
Ju. Seti , E. Vereshko ,
M. Tkach
Yuriy Fedkovych Chernivtsi National University, 2, Kotsyubynsky St., Chernivtsi, UA–58012, Ukraine
e-mail: j.seti@chnu.edu.ua
Received 03 July 2022; in final form 23 September 2022; accepted 26 September 2022; published online 22 November 2022
|
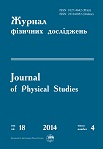 |
The study develops the theory of electron states in a multi-cascade nanostructure as the main element of a quantum cascade detector within the effective mass approximation and rectangular potentials model. Using a model with physical and geometric parameters of four-well cascades for the experimental far-infrared quantum cascade detector, the properties of the electron states and oscillator strengths were investigated, which made it possible to establish the peculiarities of the formation of the device absorption band.
It is established that in a multi-cascade structure, unlike a single-cascade model, discrete energy bands appear for the electron, where the number of levels is equal to the number of cascades. If the number of cascades increases within the range of small values ($\sim10$ cascades), the bands widths increase, and with a further increase in the number of cascades, they change insignificantly. The properties of the electron states wave functions both for the same band and for different bands are fundamentally different, since the distributions of their probability in the cascades of the structure are also different. It is shown that in a multi-cascade element of a quantum cascade detector, separate cascades or even groups of cascades can occur for each electron state, in which the probability of electron location is negligible. When the total number of cascades in the structure increases, the number of cascades in which the probability of electron location is close to zero only increases.
Calculation of oscillator strengths proves that in the $N$-cascade structure there are $N$ far-infrared quantum transitions between states, whose localization regions maximally coincide in the multi-cascade element of a quantum cascade detector. The oscillator strengths of all $N$ transitions coincide with each other and with the oscillator strength in the single-cascade model with accuracy to the second sign. As a result, in the absorption spectrum of the multi-cascade structure, a narrow band appears; its width is determined by the widths of the two electronic bands between which transitions occur.
Key words: nanostructure, electron, quantum cascade detector.
Full text
References
- M. A. Belkin, F. Capasso, Phys. Scr. 90, 118002 (2015);
Crossref
- Y. Matsuoka, M. P. Semtsiv, W. Ted Masselink, Woodhead Publishing Series in Electronic and Optical Materials, p. 131 (2020);
Crossref
- F. R. Giorgetta et al., IEEE J. Quantum Electron. 45, 1039 (2009);
Crossref
- A. Delga, Woodhead Publishing Series in Electronic and Optical Materials, p. 337 (2020);
Crossref
- J. Faist et al., Science 264, 553 (1994);
Crossref
- S. Kumar, B. S. Williams, Q. Hu, Appl. Phys. Lett. 88, 121123 (2006);
Crossref
- F. Castellano et al., J. Appl. Phys. 109, 102407 (2011);
Crossref
- S. Katz, A. Vizbaras, R. Meyer, M.-C. Amann, J. Appl. Phys. 109, 081101 (2011);
Crossref
- D. Heydari, Y. Bai, N. Bandyopadhyay, S. Slivken, M. Razeghi, Appl. Phys. Lett. 106, 091105 (2015);
Crossref
- A. Dunn et al., Nature Commun. 11 (2020);
Crossref
- L. Gendron, M. Carras, A. Huynh, V. Ortiz, Appl. Phys. Lett. 85, 2824 (2004);
Crossref
- B. F. Levine, J. Appl. Phys. 74, R1 (1993);
Crossref
- H. Schneider, H. C. Liu, Quantum Well Infrared Photodetectors, p. 1 (2007);
Crossref
- P. Reininger et al., Appl. Phys. Lett. 105, 091108 (2014);
Crossref
- J. Liu et al., Semicond. Sci. Technol. 33 125016 (2018);
Crossref
- Z. Shen et al., Appl. Phys. Lett. 118, 081102 (2021);
Crossref
- T. Dougakiuchi, A. Ito, M. Hitaka, K. Fujita, M. Yamanishia, Appl. Phys. Lett. 118, 041101 (2021);
Crossref
- L. Gendron, C. Koeniguer, V. Berger, X. Marcadet, Appl. Phys. Lett. 86, 121116 (2005);
Crossref
- F. R. Giorgetta et al., Appl. Phys. Lett. 90, 231111 (2007);
Crossref
- A. Vardi et al., Appl. Phys. Lett. 92, 011112 (2008);
Crossref
- A. Vardi et al., Appl. Phys. Lett. 93, 193509 (2008);
Crossref
- S. Sakr et al., Appl. Phys. Lett. 102, 011135 (2013);
Crossref
- Y. Song et al., Appl. Phys. Lett. 105, 182104 (2014);
Crossref
- M. Beeler, E. Trichas, E. Monroy, Semicond. Sci. Technol. 28, 074022 (2013);
Crossref
- F. R. Giorgetta et al., Appl. Phys. Lett. 91, 111115 (2007);
Crossref
- M. Giparakis et al., Appl. Phys. Lett. 120, 071104 (2022);
Crossref
- A. P. Ravikumar, T. A. Garcia, J. De Jesus, M. C. Tamargo, C. F. Gmachl, Appl. Phys. Lett. 105, 061113 (2014);
Crossref
- A. Jollivet et al., Appl. Phys. Lett. 113, 251104 (2018);
Crossref
- W. Lei, C. Jagadish, J. Appl. Phys. 104, 091101 (2008);
Crossref
- C. Koeniguer, G. Dubois, A. Gomez, V. Berger, Phys Rev B 74, 235325 (2006);
Crossref
- R. Terazzi, J. Faist, New J. Phys. 12, 033045 (2010);
Crossref
- C. Jirauschek, T. Kubis, Appl. Phys. Rev. 1, 011307 (2014);
Crossref
- G. G. Zegrya, N. V. Tkach, I. V. Boiko, Yu. A. Seti, Phys. Solid State 55, 218 (2013);
Crossref
- D. Botez, C.-C. Chang, L. J. Mawst, J. Phys. D: Appl. Phys. 49, 043001 (2016);
Crossref
- J. He et al., Appl. Phys. Express 10, 011101 (2017);
Crossref
- М. Ткаch, J. Seti, О. Voitsekhivska, Superlattices Microstruct. 109, 905 (2017);
Crossref
- S. Saha, J. Kumar, J. Appl. Phys. 121, 053104 (2017);
Crossref
- Z. Li, P. Wang, J. He, H. Chen, J. Cheng, Superlattices Microstruct. (2017);
Crossref
- J. G. Rojas-Briseño, A. Del Rio-De Santiago, M. E. Mora-Ramos, J. C. Martínez-Orozco, Optik 201, 163431 (2020);
Crossref
- Ju. Seti, O. Voitsekhivska, E. Vereshko, M. Tkach, Appl. Nanosci. 12, 533 (2022);
Crossref
- D. F. Nelson, R. C. Miller, D. A. Kleinman, Phys. Rev. B 35, 7770 (1987);
Crossref
- G. Bastard, Phys. Rev. B 24, 5693 (1981);
Crossref
- G. Bastard, Phys. Rev. B 25, 7584 (1982);
Crossref
- C.-S. Jia, L.-Z. Yi, Y. Sun, J. Math. Chem. 43, 435 (2008);
Crossref
- V. M. Tkachuk, O. O. Voznyak, Eur. Phys. J. Plus. 130, 161 (2015);
Crossref
- H. Hassanabadi, W. S. Chung, S. Zare, M, Alimohammadi, Eur. Phys. J. Plus. 132, 135 (2017);
Crossref
- I. Galbraith, G. Duggan, Phys. Rev. B 38, 10057 (1988);
Crossref
- G. T. Einevoll, P. C. Hemmer, Semicond. Sci. Technol. 6, 590 (1991);
Crossref
- D. J. BenDaniel, C. B. Duke, Phys. Rev. 152, 683 (1966);
Crossref
- P. Harrison, A. Valavanis, Quantum Wells, Wires and Dots: Theoretical and Computational Physics of Semiconductor Nanostructures, 4th ed. (Wiley, 2016);
Crossref
- J. H. Davies, The Physics of Low-dimensional Semiconductors (Cambridge University Press, 1997);
Crossref